Antidepressants as Endocrine Disrupting Compounds in Fish (2022)
MINI REVIEW article
Antidepressants as Endocrine Disrupting Compounds in Fish
- Department of Biological Sciences, University of Calgary, Calgary, AB, Canada
As antidepressant usage by the global population continues to increase, their persistent detection in aquatic habitats from municipal wastewater effluent release has led to concerns of possible impacts on non-target organisms, including fish. These pharmaceuticals have been marketed as mood-altering drugs, specifically targeting the monoaminergic signaling in the brain of humans. However, the monoaminergic systems are highly conserved and involved in the modulation of a multitude of endocrine functions in vertebrates. While most studies exploring possible impact of antidepressants on fish have focused on behavioural perturbations, a smaller spotlight has been placed on the endocrine functions, especially related to reproduction, growth, and the stress response. The purpose of this review is to highlight the possible role of antidepressants as endocrine disruptors in fish. While studies linking the effects of environmentally relevant levels of antidepressant on the endocrine system in fish are sparse, the emerging evidence suggests that early-life exposure to these compounds have the potential to alter the developmental programming of the endocrine system, which could persist as long-term and multigenerational effects in teleosts.
Introduction
Antidepressants in aquatic environments have persisted for decades and are continually introduced into our waterways through municipal wastewater effluent (MWWE) release (1). Aquatic organisms, including fish, inhabiting these waterways are persistently exposed to these compounds, but effects on non-target organisms are only beginning to emerge (2, 3). Over the last few decades, studies have examined the effects of antidepressants on teleosts, but the majority of work have focused on behavioural alterations (4–6). Monoamines, including serotonin, noradrenaline, and dopamine, are the main targets by which the antidepressants elicit a behavioural response (7). However, monoamines play significant roles in modulating other endocrine functions, including steroid hormone synthesis and action (8–13), suggesting wider impact on the physiology of fishes due to antidepressant exposures. As recent reviews have discussed the impact of antidepressants on behavioral consequences (5, 6), this minireview will be limited to the endocrine-related perturbations in fish. Here, we highlight the emerging evidence of antidepressants as endocrine disrupting compounds in fish.Antidepressants in the Aquatic Environment
Since the use of anti-histaminergic compounds in the early 1950’s, the practice of neuroactive compounds to treat behavioural deficits associated with major depressive disorder has grown (14–16). The advent of selective serotonin reuptake inhibitors (SSRI) reshaped the antidepressant market, and prescriptions increased rapidly in the early 1990s, especially with the release of fluoxetine (Prozac), citalopram (Celexa), fluvoxamine (Luvox), paroxetine (Paxil), and sertraline (Zoloft; 17). To diminish the side-effects associated with the SSRI class of compounds, additional antidepressants targeting the inhibition of reuptake of either noradrenaline (NRI), such as reboxetine (Edronax), or both serotonin and noradrenaline (SNRI), such as venlafaxine (Effexor) and duloxetine (Cymbalta), emerged (17). In humans, antidepressants typically undergo first-pass metabolism in the liver (18), and in many cases their metabolites, including norfluoxetine (fluoxetine), O-desmethylvenlafaxine (venlafaxine), and desmethylcitalopram (citalopram), remain equipotent to the parent compound (19). Consequently, excretion of these drugs, and the incomplete removal by wastewater treatment processes, has led to the antidepressants, including citalopram, sertraline, venlafaxine, fluoxetine, and other neuroactive compounds, being prevalent in MWWE at concentrations greater than parts per billion (20–27). Also, given their continued release into the environment and their slower breakdown, antidepressants are being touted as persistent contaminants in the aquatic environment (28). Given that antidepressants consumption has gone up in the last 20 years and is only expected to increase (29, 30), environmental levels will also increase in our waterways receiving MWWE outfalls. Consequently, understanding the impact of these drugs on non-target animals, such as fishes, and deciphering their mode of action leading to the adverse effects, will allow for developing environmental risk assessment models for antidepressants in our waterways.
Antidepressant Effects on Monoamine Content in Fish
In humans, antidepressants primarily target the transporters of monoamines, including serotonin, noradrenaline, and dopamine, inhibiting the reuptake of monoamines from the synaptic cleft into the presynaptic cell (6, 31). This increases the duration of exposure, as well as the concentration of monoamines in the synapse, leading to increased interaction with receptors on the postsynaptic terminal for therapeutic benefits (31, 32). In teleosts, studies have demonstrated that antidepressants interact with the monoamine transporters, and disrupt brain monoamines content (33–41). For instance, both fluoxetine and venlafaxine reduced brain serotonin levels in adult hybrid striped bass (Morone saxatilis; 33, 37, 38) and Siamese fighting fish (Betta splendens; 35). Also, exposures to venlafaxine elevated serotonin, noradrenaline, and dopamine contents in a region-specific manner in the midbrain of juvenile rainbow trout (Oncorhynchus mykiss; 36). Early developmental exposure to venlafaxine depleted serotonin immunoreactivity in the larval zebrafish (Danio rerio) brain and reduced catecholamine cell populations (39). These results suggest that antidepressants impact on brain monoamine content in fishes may be species- and/or life-stage-specific (6, 13), but a clear cause and effect relationship has not been established.
Antidepressants Effect on the Endocrine Systems in Fish
The monoaminergic system is highly conserved in vertebrates, and the central and peripheral monoamines play an important role in modulating endocrine functions (3, 8–10, 12, 42–49). Consequently, disruptions in the monoaminergic pathway is a potential target for antidepressants effect on the endocrine system (6, 13). Very little is known about the mode of action of antidepressants in disrupting the endocrine axes in teleosts. Here we will outline some of the studies that suggest that the antidepressants are endocrine disruptors, especially pertaining to reproduction, growth, and the stress response in fish.
Reproduction
Reproduction is primarily under the control of the hypothalamic-pituitary-gonadal (HPG) axis in fish (9, 50). The hypothalamus releases gonadotropin releasing hormone (GnRH), the primary endocrine signal for the release of the pituitary gonadotropins, including the follicular stimulating hormone (FSH) and luteinizing hormone (LH), into the circulation (50, 51). The actions of LH and FSH stimulates the secretion of the sex steroids, including androgens and estrogens/progestins, essential for spermatogenesis and oogenesis, respectively (52). There is evidence that antidepressants, both SSRI’s and the SNRI’s, affect reproductive performance in fish (see Table 1 for specific experimental information). For instance, fluoxetine exposure for 100 d delayed the onset of sexual maturation in the male mosquitofish (Gambusia affinis; 53). Also, fluoxetine exposure for over 7 d increased estrogen level, while reducing testosterone level and the milt volume in the male goldfish (Carassius auratus; 55). Citalopram exposure reduced whole-brain GnRH transcripts and disrupted spermatogenesis in male zebrafish (60). In females, fluoxetine exposure reduced estradiol levels in the ovary, and this correlated with a drop in egg production in zebrafish (56). A similar result was also seen in goldfish, with fluoxetine injection leading to significant reductions in circulating estradiol levels (54). Venlafaxine exposure also reduced egg production in adult zebrafish (78). Although these studies suggest that both the SSRI and the SNRI class of antidepressants have the potential to affect fish reproduction, the concentrations utilized in these studies were high and not environmentally realistic. Few studies have assessed reproductive parameters in fish upon exposure to environmentally relevant concentrations. For instance, fluoxetine (0.1 ug/L) exposure for 4 weeks perturbed estradiol levels, but did not affect the reproductive performance in the female Japanese medaka (Oryzias latipes; 59). Also, exposures to environmentally relevant concentrations of fluoxetine (30 or 380 ng/L) increased total sperm count (58), but did not affect sperm characteristics (79) in the Eastern mosquitofish (Gambusia holbrooki). These studies suggest that although exposure of adult fish to environmentally relevant levels may cause endocrine disruption, it did not appear to pose a negative risk to the reproductive outcomes. Life stage of exposure must also be considered, as growing evidence from recent studies reveal that early-life stages may be particularly sensitive to antidepressants exposure and may lead to longer-term reproductive impairment. For instance, life-long exposure to fluoxetine (0.5 or 5 µg/L) in turquoise killifish (Nothobranchius fuzeri) increased fecundity (57). Also, venlafaxine over the entire life cycle at concentrations as low as 0.88 µg/L reduced the length of the ovipositor in female fathead minnows (Pimephales promelas), while at 88 µg/L there was an increase in the total egg output of females (61). These latter studies highlight two critical lessons from contaminant studies: i) species residing in aquatic habitats receiving MWWE may be sensitive to these compounds at environmentally relevant levels, and ii) that early-life exposure windows may be more sensitive to antidepressant impact, leading to long-term effects on reproductive performance. One possible route for early exposure may be through maternal transfer of the compounds to the oocytes, especially given the bioaccumulation potential of the antidepressants in tissues including gonads (6, 59, 78, 80, 81). The mechanism(s) by which antidepressants may lead to changes in the developmental programming of the reproductive axis remains to be examined.
Although the mode of action of antidepressants in affecting reproductive endocrine disruption is unclear, one possible route may be through the modulation of the monoaminergic system. Indeed, the functioning of the hypothalamic-pituitary-gonadal (HPG) axis in fish (9, 50, 82, 83) is modulated by monoamines, including serotonin, norepinephrine, and dopamine (46, 47, 52). For example, dopamine plays an inhibitory role in fish reproduction by inhibiting gonadotropin releasing hormone (GnRH) from the hypothalamus and gonadotropins from the pituitary (48). Similarly, serotonin stimulation also regulates HPG axis (12), including stimulation of GnRH at the hypothalamus (84), and stimulation of oocyte maturation locally in the gonads by increased synthesis of estrogen and maturation-inducing steroids (85). The monoamines may also play differing roles at specific developmental stages or across species. For instance, injections of tyrosine and tryptophan (precursors of dopamine and serotonin, respectively) for 10 days in Gulf killifish (Fundulus grandis) increases gonadosomatic index in males (86). Conversely, in mummichog (Fundulus heteroclitus), serotonin exposure inhibits oocyte maturation during the follicular development stage via activation of the cAMPK-PKA transduction pathway (87, 88). Taken together, alterations in monoamine levels may be a possible mechanism by which antidepressants impact reproductive endocrine disruption, but these effects may be species-, life stage- and sex-dependent. Studies are clearly warranted with environmentally relevant species using environmentally relevant concentrations to understand the risks posed on the reproductive performance of fish.
Growth
Growth in fish is regulated through a variety of physiological pathways mediating energy acquisition and balance (89, 90). The drive to feed is regulated by the neuropeptides (reviewed by 91), with the somatotropic axis playing a key role in modulating growth and metabolism of skeletal muscle, which comprises >50% of the fish mass (89). Environmental exposures to antidepressants have been shown to restrict growth in fishes, including goldfish (64), zebrafish (66, 70, 71), meager (Argyrosomus ostregius; 65), yellow catfish (Tachysurus fulvidraco; 68), short-lived killifish (57), and fathead minnows (67, 69, 72; See Table 1 for specific experimental information). This is an important consideration, as restriction on growth is true of exposures to both the SSRI (fluoxetine, sertraline) and the SNRI class (venlafaxine), and has been noted even at environmentally relevant concentrations (68, 71, 72). Exposure during critical developmental windows appears to be more sensitive, as early-life (72) and zygotic (70) exposures led to growth retardations at later life-stages. These growth effects may be related to possible endocrine disruptions. A key aspect of growth is the endocrine control of feeding and metabolism (89, 90). Antidepressants exposure have been shown to reduce feeding responses in fish (92), including goldfish (64), stickleback (Gasterosteus aculeatus; 93), fathead minnows (94, 95), rainbow trout (36), hybrid striped bass (37, 38, 96), mosquito fish (79), and European perch (Perca fluviatlis; 92). As the drive to feed is regulated by the expression of neuropeptides (reviewed by 91), it is possible that these peptides are targets for the impact of the drug. For instance, antidepressants have been shown to influence several key genes involved in feeding activity in fish, including proopiomelanocortin b, corticotropin releasing hormone (CRH), and neuropeptide Y, and these changes correlated with reduced food intake (36, 64, 68), which may be reflected in reduced growth rates. The growth hormone (GH) and the insulin-like growth factors (IGFs), and their receptors and binding proteins are all key components of the somatotropic axis, orchestrating growth and metabolism in fish (97–99). The functioning of the somatotropic axis is regulated by monoaminergic stimulation in fish (10, 100). For instance, serotonin stimulation increases GH secretion (101), while catecholamines, including norepinephrine reduce GH secretion (102). Dopamine also pays a role in modulating GH release in fish (reviewed by 8). For instance, exposure to L-DOPA (precursor of dopamine), dopamine, or D1/D2 receptor agonists (apomorphine) by intraperitoneal injection increased the serum levels of GH in goldfish (102). This increase in GH appears to be driven primarily by the activation of the D1 receptor (103), and involves the cAMP-PKA pathway (104). We have also previously shown that venlafaxine reduced the transcript abundances of growth hormone receptors, IGF2, leptin a, the leptin receptor, and myostatin, in the liver of zebrafish, possibly signaling a disruption in the growth axis functioning, and also leading to metabolic disruption (70). A similar effect was also seen following sertraline exposure in the yellow catfish, experiencing reductions in somatostatin, growth hormone, igf, and neuropeptide y transcript abundance in the brain (68). Together, given the modulatory role of monoamines on the growth axis, it is likely that alterations to their levels due to antidepressant exposure has the potential to impact the feeding and growth performance of fish. Importantly, our study demonstrated that an early-life exposure to venlafaxine can lead to long-term perturbations in the programming of the growth axis (70), but whether this occurs at environmental levels of the antidepressant remain unexplored.
Corticosteroid Stress Response
The endocrine stress response is highly conserved in vertebrates and is essential to allow animals to cope with stress (105–109). The primary stress response includes the sympathetic nervous system activation leading to the release of catecholamines, and this is followed by the release of the corticosteroids from the adrenal glands in mammals and the interrenal tissue in teleosts (105, 110–112). In fish, cortisol is the major corticosteroid released in response to stress and its release is mediated by the activation of the hypothalamus-pituitary-interrenal (HPI) axis (106, 107, 111, 113). Once released into the circulation, cortisol action in target tissues is mediated by the activation of either the glucocorticoid receptor (GR) and/or the mineralocorticoid receptor (MR; 114–116). Monoamines play an important role in the regulation of the corticosteroid stress axis (117, 118). In fish, studies have demonstrated that serotonin influences downstream cortisol production (119, 120). For example, intraperitoneal injection of 8-OH-DPAT, a 5HT1A receptor agonist, elevates basal levels of cortisol, and inhibits the attendant rise in cortisol following an acute stressor in juvenile Arctic charr (Salvelinus alpinus; 121). This was also mimicked by increased intake of tryptophan, which counteracted the stress-induced elevations in plasma cortisol in rainbow trout (122). Chromaffin cells of the head kidney have been shown to contain serotonin, and this monoamine is capable of stimulating the release of cortisol from interrenal tissues in vitro (123). Catecholamines can also exert influence on the stress axis function, with noradrenaline capable of stimulating CRH secretion in tilapia in vitro (124), and the injection of noradrenaline and adrenaline stimulating the release of cortisol in sea bass (Dicentrarchus labrax; 125). Dopamine, in contrast has been shown to inhibit cortisol release (121), and this may occur even at the level of the pituitary (126). Consequently, the monoaminergic system exerts control over the activity of the HPI axis and may be a target for the antidepressant impact on the stress axis in fish.
Investigations of possible impacts to the stress axis in fish by antidepressants have primarily been carried out using fluoxetine and venlafaxine, with recent evidence suggesting impacts may be sex-specific (See Table 1 for specific experimental information). The responses seen in the activity of the stress axis appears to be life stage-specific in fish. For instance, the circulating cortisol levels increase following an intraperitoneal administration of 50 µg/g of fluoxetine in the adult Gulf toadfish (Opsanus beta) (73). Also, juvenile rainbow trout exposed to environmental levels of venlafaxine (0.1 and 1 ug/L) display amplified cortisol responses following a social stressor, and this correlated with increases in the transcript abundance of crh and pomcb in the brain. Conversely, antidepressants exposure to early-life stages suppressed the stress-induced cortisol production, and also led to long-term impacts on cortisol response, suggesting alterations in the developmental programming of the stress axis (74, 75, 77). For instance, early life exposure to fluoxetine (either 0.54 µg/L or 54 µg/L) reduces basal and stressed levels of cortisol in zebrafish (75). When these fish grew to adults, the attenuated stressor-induced cortisol response was sexually dimorphic with the males more sensitive than females (75, 127). In contrast, zygotic deposition of venlafaxine did not influence cortisol levels at the larval stage, but the stressor-induced cortisol production was attenuated in the female and not male fish as adults (77). Vera-Chang and colleagues (75) suggested that disruptions at the level of the head kidney limited cortisol production in response to fluoxetine, while venlafaxine exposure revealed that the head kidney was still responsive to ACTH (75), suggesting that the disruption in cortisol production was at the level of the hypothalamus and/or pituitary. Although the route of exposure and duration of exposure were different between the two antidepressants (75, 77, 127), the results suggest that the mode of action in affecting the stress axis functioning may be distinct. At least in zebrafish, sex-specific differences in adrenoreceptors are seen in females relative to males, particularly in the hypothalamus and pre-optic areas, with female fish exhibiting higher levels of α2 adrenoreceptors (128). It remains to be seen if the difference in sex-specific effect seen due to early life exposure to either fluoxetine or venlafaxine may be related to their effect on the reuptake of serotonin or serotonin and norepinephrine, respectively. Taken together, these studies demonstrate that the class of antidepressants and duration of exposure may influence the outcome of the endocrine disruption of the stress axis in a sex-specific manner. Regardless, disruptions in cortisol levels may compromise their stress coping capability, as cortisol and the concomitant activation of GR and/or MR are integral players in the energy substrate partitioning to cope with stress (114–116, 129). The mechanisms leading to antidepressants effect on the stress axis warrant further study, especially given that some of these changes are even passed on to successive generations (63, 74, 75, 77, 127). This leads to the proposal that epigenetic changes due to early life exposure to the antidepressants may be involved in the long-term programming of the stress axis in teleosts.
Conclusions and Future Directions
Antidepressants alter central monoamine levels, and this may play a role in the behavioural perturbations in fish (6, 39, 128). The emerging evidence also points to antidepressants as endocrine disrupting compounds in fish (Table 1). Specifically, studies reveal that the antidepressants impact the endocrine control of growth, stress, and reproductive axes in fish (Figure 1). As the monoaminergic system modulates endocrine function, a possible mode of action of the antidepressants in affecting endocrine disruption may be through alterations in the central and peripheral monoamine content, but this remains to be established. It is particularly important to note that early life exposures to antidepressants impacts the developmental programming of the endocrine system, and this may be reflected in long term and multigenerational changes in hormone levels and function (75, 77).We recently showed that venlafaxine-mediated disruption in embryonic serotonin content alters neurogenesis (39), and whether this alteration in brain development may play a role in the developmental programming of the endocrine axes warrants further study. For instance, the availability of knockout models in fish, including tryptophan hydroxylase paralogs (92, 129), a rate limiting enzyme in serotonin synthesis, will be an important step in delineating the effects of antidepressants mediated by the serotonergic pathway. While targeting the changes in the monoamine content may be one possible mechanism leading to endocrine disruption, the possibility that antidepressants may have direct effect on hormone action by targeting receptor function cannot be excluded (62). The sex of the animal is also another key factor that may dictate the outcome of the antidepressants impact (73, 75, 77, 127), and should be taken into account in future studies on endocrine disruption. Elucidating the mechanism of action of antidepressants in disrupting endocrine function in non-target organisms would be an important future direction to assess their impact on fitness consequences to the animal. A major limitation of the current body of work is the scarcity of studies on the endocrine effects related to environmentally relevant concentrations of the antidepressants in species commonly found in the aquatic habitats receiving MWWE. Environmental disruptors, including antidepressants are persistent in the aquatic habitats, suggestive of life-long exposures to these compounds (28). However, the implications of such life long exposures on animal fitness, and multigenerational consequences await further study. Such studies would be essential in developing markers for environmental risk assessment and for establishing adverse outcome pathways related to endocrine disruptive impacts of antidepressants. From a mechanistic standpoint, future studies should aim to identify the critical window during early development that is most sensitive to antidepressants and understand the mechanisms of action by which these pharmaceuticals alter the developmental programming of the endocrine system. This would allow for the development of novel biomarkers that are indicative of the developmental origin of endocrine dysfunction due to antidepressants exposure in fish.
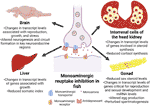
Figure 1 Overview of the tissue-specific impacts of antidepressants on the endocrine axes in fish. Depiction of the expected mode of action of antidepressants, along with the specific tissues and the observed phenotypes associated with exposures to antidepressants.
Author Contributions
All authors listed have made a substantial, direct, and intellectual contribution to the work and approved it for publication.
Funding
The authors thank the funding support from the Natural Sciences and Engineering Research Council of Canada Discovery Grant awarded to MMV (RGPIN-2019-06291).
Conflict of Interest
The authors declare that the research was conducted in the absence of any commercial or financial relationships that could be construed as a potential conflict of interest.
Publisher’s Note
All claims expressed in this article are solely those of the authors and do not necessarily represent those of their affiliated organizations, or those of the publisher, the editors and the reviewers. Any product that may be evaluated in this article, or claim that may be made by its manufacturer, is not guaranteed or endorsed by the publisher.
References
1. Daughton CG, Ternes TA. Pharmaceuticals and Personal Care Products in the Environment: Agents of Subtle Change? Environ Health Perspect (1999) 107:907–38. doi: 10.1289/ehp.99107s6907
2. Söffker M, Tyler CR. Endocrine Disrupting Chemicals and Sexual Behaviors in Fish a Critical Review on Effects and Possible Consequences. Crit Rev Toxicol (2012) 42:653–68. doi: 10.3109/10408444.2012.692114
3. Mennigen JA, Stroud P, Zamora JM, Moon TW, Trudeau VL. Pharmaceuticals as Neuroendocrine Disruptors: Lessons Learned From Fish on Prozac. J Toxicol Environ Heal - Part B Crit Rev (2011) 14:387–412. doi: 10.1080/10937404.2011.578559
4. Melvin SD. Effect of Antidepressants on Circadian Rhythms in Fish : Insights and Implications Regarding the Design of Behavioural Toxicity Tests. Aquat Toxicol (2017) 182:20–30. doi: 10.1016/j.aquatox.2016.11.007
5. Sehonova P, Svobodova Z, Dolezelova P, Vosmerova P, Faggio C. Effects of Waterborne Antidepressants on non-Target Animals Living in the Aquatic Environment: A Review. Sci Total Environ (2018) 631–632:789–94. doi: 10.1016/j.scitotenv.2018.03.076
6. Gould SL, Winter MJ, Norton WHJ, Tyler CR. The Potential for Adverse Effects in Fish Exposed to Antidepressants in the Aquatic Environment. Environ Sci Technol (2021) 55:16299–312. doi: 10.1021/acs.est.1c04724
7. Hillhouse TM, Porter JH. A Brief History of the Development of Antidepressant Drugs: From Monoamines to Glutamate. Exp Clin Psychopharmacol (2015) 23:1–21. doi: 10.1037/a0038550.A
8. Peng C, Peter RE. Neuroendocrine Regulation of Growth Hormone Secretion and Growth in Fish. Zool Stud (1997) 36:79–89.
9. Trudeau VL. Neuroendocrine Regulation of Gonadotrophin II Release and Gonadal Growth in the Goldfish, Camssius Auratns. Rev Reprod (1997) 2:55–68. doi: 10.1530/revreprod/2.1.55
10. Canosa LF, Chang JP, Peter RE. Neuroendocrine Control of Growth Hormone in Fish. Gen Comp Endocrinol (2007) 151:1–26. doi: 10.1016/j.ygcen.2006.12.010
11. Kreke N, Dietrich DR. Physiological Endpoints for Potential SSRI Interactions in Fish. Crit Rev Toxicol (2008) 38:215–47. doi: 10.1080/10408440801891057
12. Prasad P, Ogawa S, Parhar IS. Role of Serotonin in Fish Reproduction. Front Neurosci (2015) 9:195. doi: 10.3389/fnins.2015.00195
13. McDonald MD. An AOP Analysis of Selective Serotonin Reuptake Inhibitors (SSRIs) for Fish. Comp Biochem Physiol Part - C Toxicol Pharmacol (2017) 197:19–31. doi: 10.1016/j.cbpc.2017.03.007
14. Huang H, Coleman S, Bridge JA, Yonkers K, Katon W. A Meta-Analysis of the Relationship Between Antidepressant Use in Pregnancy and the Risk of Preterm Birth and Low Birth Weight. Gen Hosp Psychiatry (2014) 36:13–8. doi: 10.1016/j.genhosppsych.2013.08.002
15. Mars B, Heron J, Kessler D, Davies NM, Martin RM, Thomas KH, et al. Influences on Antidepressant Prescribing Trends in the UK: 1995–2011. Soc Psychiatry Psychiatr Epidemiol (2017) 52:193–200. doi: 10.1007/s00127-016-1306-4
16. Pratt LA, Brody DJ, Gu Q. Antidepressant Use Among Persons Aged 12 and Over:United States,2011-2014. NCHS Data Brief (2017) 283:1–8.
17. Pereira VS, Hiroaki-Sato VA. A Brief History of Antidepressant Drug Development: From Tricyclics to Beyond Ketamine. Acta Neuropsychiatr (2018) 30:307–22. doi: 10.1017/neu.2017.39
18. Telles-Correia D, Barbosa A, Cortez-Pinto H, Campos C, Rocha NBF, Machado S. Psychotropic Drugs and Liver Disease: A Critical Review of Pharmacokinetics and Liver Toxicity. World J Gastrointest Pharmacol Ther (2017) 8:26. doi: 10.4292/wjgpt.v8.i1.26
19. Owens MJ, Morgan WN, Plott SJ, Nemeroff CB. Neurotransmitter Receptor and Transporter Binding Profile of Antidepressants and Their Metabolites. J Pharmacol Exp Ther (1997) 283:1305–22.
20. Kolpin DW, Furlong ET, Meyer MT, Thurman EM, Zaugg SD, Barber LB, et al. Pharmaceuticals, Hormones, and Other Organic Wastewater Contaminants in U.S. Streams, 1999-2000: A National Reconnaissance. Environ Sci Technol (2002) 36:1202–11. doi: 10.1021/es011055j
21. Archer E, Petrie B, Kasprzyk-Hordern B, Wolfaardt GM. The Fate of Pharmaceuticals and Personal Care Products (PPCPs), Endocrine Disrupting Contaminants (EDCs), Metabolites and Illicit Drugs in a WWTW and Environmental Waters. Chemosphere (2017) 174:437–46. doi: 10.1016/j.chemosphere.2017.01.101
22. Gasser G, Pankratov I, Elhanany S, Werner P, Gun J, Gelman F, et al. Field and Laboratory Studies of the Fate and Enantiomeric Enrichment of Venlafaxine and O-Desmethylvenlafaxine Under Aerobic and Anaerobic Conditions. Chemosphere (2012) 88:98–105. doi: 10.1016/j.chemosphere.2012.02.074
23. Schlüsener MP, Hardenbicker P, Nilson E, Schulz M, Viergutz C, Ternes TA. Occurrence of Venlafaxine, Other Antidepressants and Selected Metabolites in the Rhine Catchment in the Face of Climate Change. Environ Pollut (2015) 196:247–56. doi: 10.1016/j.envpol.2014.09.019
24. Fernández-Rubio J, Rodríguez-Gil JL, Postigo C, Mastroianni N, López de Alda M, Barceló D, et al. Psychoactive Pharmaceuticals and Illicit Drugs in Coastal Waters of North-Western Spain: Environmental Exposure and Risk Assessment. Chemosphere (2019) 224:379–89. doi: 10.1016/j.chemosphere.2019.02.041
25. Papageorgiou M, Kosma C, Lambropoulou D. Seasonal Occurrence, Removal, Mass Loading and Environmental Risk Assessment of 55 Pharmaceuticals and Personal Care Products in a Municipal Wastewater Treatment Plant in Central Greece. Sci Total Environ (2016) 543:547–69. doi: 10.1016/j.scitotenv.2015.11.047
26. Metcalfe CD, Chu S, Judt C, Li H, Oakes KD, Servos MR, et al. Antidepressants and Their Metabolites in Municipal Wastewater, and Downstream Exposure in an Urban Watershed. Environ Toxicol Chem (2010) 29:79–89. doi: 10.1002/etc.27
27. Rúa-Gómez PC, Püttmann W. Impact of Wastewater Treatment Plant Discharge of Lidocaine, Tramadol, Venlafaxine and Their Metabolites on the Quality of Surface Waters and Groundwater. J Environ Monit (2012) 14:1391. doi: 10.1039/c2em10950f
28. Brooks BW. Fish on Prozac (and Zoloft): Ten Years Later. Aquat Toxicol (2014) 151:61–7. doi: 10.1016/j.aquatox.2014.01.007
29. Rabeea SA, Merchant HA, Khan MU, Kow CS, Hasan SS. Surging Trends in Prescriptions and Costs of Antidepressants in England Amid COVID-19. DARU J Pharm Sci (2021) 29:217–21. doi: 10.1007/2Fs40199-021-00390-z
30. Schifano F, Chiappini S. Is There a Potential of Misuse for Venlafaxine and Bupropion? Front Pharmacol (2018) 9:239. doi: 10.3389/fphar.2018.00239
31. Harvey AT, Rudolph RL, Preskorn SH. Evidence of the Dual Mechanisms of Action of Venlafaxine. Arch Gen Psychiatry (2000) 57:503–9. doi: 10.1001/archpsyc.57.5.503
32. Feighner JP. Mechanism of Action of Antidepressant Medications R Py Co Ig a Du Es R Co Ht Ig Es in. J Clin Psychiatry (1999) 60:4–11.
33. Gaworecki KM, Klaine SJ. Behavioral and Biochemical Responses of Hybrid Striped Bass During and After Fluoxetine Exposure. Aquat Toxicol (2008) 88:207–13. doi: 10.1016/j.aquatox.2008.04.011
34. Winder VL, Sapozhnikova Y, Pennington PL, Wirth EF. Effects of Fluoxetine Exposure on Serotonin-Related Activity in the Sheepshead Minnow (Cyprinodon Variegatus) Using LC/MS/MS Detection and Quantitation. Comp Biochem Physiol - C Toxicol Pharmacol (2009) 149:559–65. doi: 10.1016/j.cbpc.2008.12.008
35. Clotfelter ED, O’Hare EP, McNitt MM, Carpenter RE, Summers CH. Serotonin Decreases Aggression via 5-HT1A Receptors in the Fighting Fish Betta Splendens. Pharmacol Biochem Behav (2007) 87:222–31. doi: 10.1016/j.pbb.2007.04.018
36. Melnyk-Lamont N, Best C, Gesto M, Vijayan MM. The Antidepressant Venlafaxine Disrupts Brain Monoamine Levels and Neuroendocrine Responses to Stress in Rainbow Trout. Environ Sci Technol (2014) 48:13434–42. doi: 10.1021/es504331n
37. Bisesi JH, Bridges W, Klaine SJ. Effects of the Antidepressant Venlafaxine on Fish Brain Serotonin and Predation Behavior. Aquat Toxicol (2014) 148:130–8. doi: 10.1016/j.aquatox.2013.12.033
38. Bisesi JH, Sweet LE, van den Hurk P, Klaine SJ. Effects of an Antidepressant Mixture on the Brain Serotonin and Predation Behavior of Hybrid Striped Bass. Environ Toxicol Chem (2015) 35:938–45. doi: 10.1002/etc.3114
39. Thompson WA, Vijayan MM. Zygotic Venlafaxine Exposure Impacts Behavioral Programming by Disrupting Brain Serotonin in Zebrafish. Environ Sci Technol (2020) 54:14578–88. doi: 10.1021/acs.est.0c06032
40. Valenti TW Jr., Gould GG, Berninger JP, Connors KA, Keele NB, Prosser KN, et al. Human Therapeutic Plasma Levels of the Selective Serotonin Reuptake Inhibitor (SSRI) Sertraline Decrease Serotonin Reuptake Transporter Binding and Shelter-Seeking Behavior in Adult Male Fathead Minnows. Environ Sci Technol (2012) 21:2427–35. doi: 10.1021/es204164b.Human
41. Thompson WA, Arnold VI, Vijayan MM. Venlafaxine in Embryos Stimulates Neurogenesis and Disrupts Larval Behavior in Zebrafish. Environ Sci Technol (2017) 51:12889–97. doi: 10.1021/acs.est.7b04099
42. Pivonello R, Ferone D, Lombardi G, Colao A, Lamberts SWJ, Hofland LJ. Novel Insights in Dopamine Receptor Physiology. Eur J Endocrinol Suppl (2007) 156:S13-21. doi: 10.1530/eje.1.02353
43. Yabut JM, Crane JD, Green AE, Keating DJ, Khan WI, Steinberg GR. Emerging Roles for Serotonin in Regulating Metabolism: New Implications for an Ancient Molecule. Endocr Rev (2019) 40:1092–107. doi: 10.1210/er.2018-00283
44. Miller GD. Appetite Regulation: Hormones, Peptides, and Neurotransmitters and Their Role in Obesity. Am J Lifestyle Med (2019) 13:586–601. doi: 10.1177/1559827617716376
45. Soares MC, Gerlai R, Maximino C. The Integration of Sociality, Monoamines and Stress Neuroendocrinology in Fish Models: Applications in the Neurosciences. J Fish Biol (2018) 93:170–91. doi: 10.1111/jfb.13757
46. Muñoz-Cueto JA, Zmora N, Paullada-Salmeron J, Marvel M, Mananos E, Zohar Y. The Gonadotropin-Releasing Hormones: Lessons From Fish. Gen Comp Endocrinol (2020) 15:113422. doi: 10.1016/j.ygcen.2020.113422
47. Zohar Y. Fish Reproductive Biology- Reflecting on Five Decades of Fundamental and Translational Research. Gen Comp Endocrinol (2020) 300:113544. doi: 10.1016/j.ygcen.2020.113544
48. Dufour S, Sebert ME, Weltzien FA, Rousseau K, Pasqualini C. Neuroendocrine Control by Dopamine of Teleost Reproduction. J Fish Biol (2010) 76:129–60. doi: 10.1111/j.1095-8649.2009.02499.x
49. Müller EE, Locatelli V, Cocchi D. Neuroendocrine Control of Growth Hormone Secretion. Physiol Rev (1999) 79:511–607. doi: 10.1152/physrev.1999.79.2.511
50. Faught E, Vijayan MM. Maternal Stress and Fish Reproduction: The Role of Cortisol Revisited. Fish Fish (2018) 19:1016–30. doi: 10.1111/faf.12309
51. Lubzens E, Bobe J, Young G, Sullivan CV. Maternal Investment in Fish Oocytes and Eggs: The Molecular Cargo and Its Contributions to Fertility and Early Development. Aquaculture (2017) 472:107–43. doi: 10.1016/j.aquaculture.2016.10.029
52. Yaron Z, Levavi-Sivan B. Hormonal Control of Reproduction and Growth | Endocrine Regulation of Fish Reproduction. San Diego: Elsevier Inc (2011). doi: 10.1016/B978-0-12-374553-8.00058-7
53. Henry TB, Black MC. Acute and Chronic Toxicity of Fluoxetine (Selective Serotonin Reuptake Inhibitor) in Western Mosquitofish. Arch Environ Contam Toxicol (2008) 54:325–30. doi: 10.1007/s00244-007-9018-0
54. Mennigen JA, Martyniuk CJ, Crump K, Xiong H, Zhao E, Popesku J, et al. Effects of Fluoxetine on the Reproductive Axis of Female Goldfish ( Carassius Auratus). Physiol Genomics (2008) 35:273–82. doi: 10.1152/physiolgenomics.90263.2008
55. Mennigen JA, Lado WE, Zamora JM, Duarte-guterman P, Langlois VS, Metcalfe CD, et al. Waterborne Fluoxetine Disrupts the Reproductive Axis in Sexually Mature Male Goldfish , Carassius Auratus. Aquat Toxicol (2010) 100:354–64. doi: 10.1016/j.aquatox.2010.08.016
56. Lister A, Regan C, Van Zwol J, van der Kraak G. Inhibition of Egg Production in Zebrafish by Fluoxetine and Municipal Effluents: A Mechanistic Evaluation. Aquat Toxicol (2009) 95:320–9. doi: 10.1016/j.aquatox.2009.04.011
57. Thoré ESJ, Philippe C, Brendonck L, Pinceel T. Antidepressant Exposure Reduces Body Size, Increases Fecundity and Alters Social Behavior in the Short-Lived Killifish Nothobranchius Furzeri. Environ Pollut (2020) 265:115068. doi: 10.1016/j.envpol.2020.115068
58. Bertram MG, Ecker TE, Wong BBM, O’Bryan MK, Baumgartner JB, Martin JM, et al. The Antidepressant Fluoxetine Alters Mechanisms of Pre- and Post-Copulatory Sexual Selection in the Eastern Mosquitofish (Gambusia Holbrooki). Environ Pollut (2018) 238:238–47. doi: 10.1016/j.envpol.2018.03.006
59. Foran CM, Weston J, Slattery M, Brooks BW, Huggett DB. Reproductive Assessment of Japanese Medaka (Oryzias Latipes) Following a Four-Week Fluoxetine (SSRI) Exposure. Arch Environ Contam Toxicol (2004) 46:511–7. doi: 10.1007/s00244-003-3042-5
60. Prasad P, Ogawa S, Parhar IS. Serotonin Reuptake Inhibitor Citalopram Inhibits Gnrh Synthesis and Spermatogenesis in the Male Zebrafish. Biol Reprod (2015) 93:1-10. doi: 10.1095/biolreprod.115.129965
61. Parrott JL, Metcalfe CD. Assessing the Effects of the Antidepressant Venlafaxine to Fathead Minnows Exposed to Environmentally Relevant Concentrations Over a Full Life Cycle. Environ Pollut (2017) 229:403–11. doi: 10.1016/j.envpol.2017.06.009
62. Galus M, Kirischian N, Higgins S, Purdy J, Chow J, Rangaranjan S, et al. Chronic, Low Concentration Exposure to Pharmaceuticals Impacts Multiple Organ Systems in Zebrafish. Aquat Toxicol (2013) 132–133:200–11. doi: 10.1016/j.aquatox.2012.12.021
63. Ikert H, Craig PM. Chronic Exposure to Venlafaxine and Increased Water Temperature Reversibly Alters microRNA in Zebrafish Gonads (Danio Rerio). Comp Biochem Physiol - Part D (2020) 33:100634. doi: 10.1016/j.cbd.2019.100634
64. Mennigen JA, Harris EA, Chang JP, Moon TW, Trudeau VL. Fluoxetine Affects Weight Gain and Expression of Feeding Peptides in the Female Goldfish Brain. Regul Pept (2009) 155:99–104. doi: 10.1016/j.regpep.2009.01.001
65. Duarte IA, Reis-Santos P, Novais SC, Rato LD, Lemos MFL, Freitas A, et al. Depressed, Hypertense and Sore: Long-Term Effects of Fluoxetine, Propranolol and Diclofenac Exposure in a Top Predator Fish. Sci Total Environ (2020) 712:136564. doi: 10.1016/j.scitotenv.2020.136564
66. de Farias NO, Oliveira R, Moretti PNS, Pinto JME, Oliveira AC, Santos VL, et al. Fluoxetine Chronic Exposure Affects Growth, Behavior and Tissue Structure of Zebrafish. Comp Biochem Physiol Part - C Toxicol Pharmacol (2020) 237:108836. doi: 10.1016/j.cbpc.2020.108836
67. Valenti TW, Perez-Hurtado P, Chambliss CK, Brooks BW. Aquatic Toxicity of Sertraline to Pimephales Promelas at Environmentally Relevant Surface Water Ph. Environ Toxicol Chem (2009) 28:2685–94. doi: 10.1897/08-546.1
68. Chen H, Liang X, Gu X, Zeng Q, Mao Z, Martyniuk CJ. Environmentally Relevant Concentrations of Sertraline Disrupts Behavior and the Brain and Liver Transcriptome of Juvenile Yellow Catfish (Tachysurus Fulvidraco): Implications for the Feeding and Growth Axis. J Hazard Mater (2021) 409:124974. doi: 10.1016/j.jhazmat.2020.124974
69. Parrott JL, Metcalfe CD. Nest-Defense Behaviors in Fathead Minnows After Lifecycle Exposure to the Antidepressant Venlafaxine. Environ Pollut (2018) 234:223–30. doi: 10.1016/j.envpol.2017.11.049
70. Thompson WA, Vijayan MM. Venlafaxine Deposition in the Zygote Disrupts the Endocrine Control of Growth in Juvenile Zebrafish. Environ Res (2021) 202:111665. doi: 10.1016/j.envres.2021.111665
71. Tang Y, Mi P, Li M, Zhang S, Li J, Feng X. Environmental Level of the Antidepressant Venlafaxine Induces Behavioral Disorders Through Cortisol in Zebrafish Larvae (Danio Rerio). Neurotoxicol Teratol (2021) 83:106942. doi: 10.1016/j.ntt.2020.106942
72. Thompson WA, Vijayan MM. Environmental Levels of Venlafaxine Impact Larval Behavioural Performance in Fathead Minnows. Chemosphere (2020) 259:127437. doi: 10.1016/j.chemosphere.2020.127437
73. Morando MB, Medeiros LR, McDonald MD. Fluoxetine Treatment Affects Nitrogen Waste Excretion and Osmoregulation in a Marine Teleost Fish. Aquat Toxicol (2009) 93:253–60. doi: 10.1016/j.aquatox.2009.03.011
74. Vera-Chang MN, Moon TW, Trudeau V. Cortisol Disruption and Transgenerational Alteration in the Expression of Stress-Related Genes in Zebrafish larvae Following Fluoxetine Exposure. Toxicol Applied Pharmacol (2019) 382:114742. doi: 10.1016/j.taap.2019.114742
75. Vera-Chang MN, St-jacques AD, Gagné R, Martyniuk CJ, Yauk CL. Transgenerational Hypocortisolism and Behavioral Disruption are Induced by the Antidepressant Fluoxetine in Male Zebrafish Danio Rerio. Proc Natl Acad Sci (2018) 115:1–8. doi: 10.1073/pnas.1811695115
76. Vera-Chang MN, St-jacques AD, Lu C, Moon TW, Trudeau VL, Carr JA. Fluoxetine Exposure During Sexual Development Disrupts the Stress Axisand Results in Sex- and Time- Dependent Effects on the Exploratory Behavior in Adult Zebrafish Danio Rerio. Front Neurosci (2019) 13:1015. doi: 10.3389/fnins.2019.01015
77. Thompson WA, Vijayan MM. Zygotic Exposure to Venlafaxine Disrupts Cortisol Stress Axis Activity in Multiple Generations of Zebrafish. Environ Pollut (2021) 274:116535. doi: 10.1016/j.envpol.2021.116535
78. Galus M, Kirischian N, Higgins S, Purdy J, Chow J, Rangaranjan S, et al. Chronic, Low Concentration Exposure to Pharmaceuticals Impacts Multiple Organ Systems in Zebrafish. Aquat Toxicol (2013) 132–133:200–11. doi: 10.1016/j.aquatox.2012.12.021
79. Martin JM, Bertram MG, Saaristo M, Ecker TE, Hannington SL, Tanner JL, et al. Impact of the Widespread Pharmaceutical Pollutant Fluoxetine on Behaviour and Sperm Traits in a Freshwater Fish. Sci Total Environ (2019) 650:1771–8. doi: 10.1016/j.scitotenv.2018.09.294
80. McCallum ES, Krutzelmann E, Brodin T, Fick J, Sundelin A, Balshine S. Exposure to Wastewater Effluent Affects Fish Behaviour and Tissue-Specific Uptake of Pharmaceuticals. Sci Total Environ (2017) 605–606:578–88. doi: 10.1016/j.scitotenv.2017.06.073
81. Arnnok P, Singh RR, Burakham R, Pérez-Fuentetaja A, Aga DS. Selective Uptake and Bioaccumulation of Antidepressants in Fish From Effluent-Impacted Niagara River. Environ Sci Technol (2017) 51:10652–62. doi: 10.1021/acs.est.7b02912
82. Zohar Y, Muñoz-Cueto JA, Elizur A, Kah O. Neuroendocrinology of Reproduction in Teleost Fish. Gen Comp Endocrinol (2010) 165:438–55. doi: 10.1016/j.ygcen.2009.04.017
83. Trudeau VL, Spanswick D, Fraser EJ, Lariviere K, Crump D, Chiu S, et al. The Role of Amino Acid Neurotransmitters in the Regulation of Pituitary Gonadotropin Release in Fish. Biochem Cell Biol (2000) 78:241–59. doi: 10.1139/o99-075
84. Khan IA, Thomas P. Immunocytochemical Localization of Serotonin and Gonadotropin-Releasing Hormone in the Brain and Pituitary Gland of the Atlantic Croaker Micropogonias Undulatus. Gen Comp Endocrinol (1993) 91:167–80. doi: 10.1006/gcen.1993.1116
85. Iwamatsu T, Toya Y, Sakai N, Terada Y, Nagata R, Nagahama Y. Effect of 5-Hydroxytryptamine on Steroidogenesis and Oocyte Maturation in Pre-Ovulatory Follicles of the Medaka Oryzias Latipes Medaka/Steroidogenesis/5-Hydroxytryptamine/Foillicle/Oocyte Maturation. Dev Growth Differ (1993) 35:625–30. doi: 10.1111/j.1440-169X.1993.00625.x
86. Emata AC, Meier AH, Spieler RE. Temporal Variations in Gonadal and Body Fat Responses to Daily Injections of 5-Hydroxytryptophan (5-HTP) and Dihydroxyphenylalanine (DOPA) in the Gulf Killifish, Fundulus Grandis. J Exp Zool (1985) 233:29–34. doi: 10.1002/jez.1402330106
87. Cerdá J, Petrino TR, Lin YP, Wallace RA. Inhibition of Fundulus Heteroclitus Oocyte Maturation In Vitro by Serotonin (5-Hydroxytryptamine). J Exp Zool (1995) 273:224–33. doi: 10.1002/jez.1402730307
88. Cerda J, Reich G, Wallace RA, Selman K. Serotonin Inhibition of Steroid-Induced Meiotic Maturation in the Teleost Fundulus Heteroclitus: Role of Cyclic AMP and Protein Kinases. Mol Reprod Dev (1998) 49:333–41. doi: 10.1002/(SICI)1098-2795(199803)49:3<333::AID-MRD14>3.0.CO;2-X
89. Sadoul B, Vijayan MM. Stress and Growth. In: Biology of Stress in Fish, volume 35 Fish Physiology, Elsevier Inc, San Diego (2016) 35:167–205. doi: 10.1016/B978-0-12-802728-8.00005-9
90. Mommsen TP. Paradigms of Growth in Fish. Comp Biochem Physiol - B Biochem Mol Biol (2001) 129:207–19. doi: 10.1016/S1096-4959(01)00312-8
91. Volkoff H. The Neuroendocrine Regulation of Food Intake in Fish: A Review of Current Knowledge. Front Neurosci (2016) 10:540. doi: 10.3389/fnins.2016.00540
92. Hedgespeth ML, Nilsson PA, Berglund O. Ecological Implications of Altered Fish Foraging After Exposure to an Antidepressant Pharmaceutical. Aquat Toxicol (2014) 151:84–7. doi: 10.1016/j.aquatox.2013.12.011
93. Kellner M, Porseryd T, Porsch-Hällström I, Hansen SH, Olsén KH. Environmentally Relevant Concentrations of Citalopram Partially Inhibit Feeding in the Three-Spine Stickleback (Gasterosteus Aculeatus). Aquat Toxicol (2015) 158:165–70. doi: 10.1016/j.aquatox.2014.11.003
94. Stanley JK, Ramirez AJ, Chambliss CK, Brooks BW. Enantiospecific Sublethal Effects of the Antidepressant Fluoxetine to a Model Aquatic Vertebrate and Invertebrate. Chemosphere (2007) 69:9–16. doi: 10.1016/j.chemosphere.2007.04.080
95. Ii JW, Klaper R. Environmental Concentrations of the Selective Serotonin Reuptake Inhibitor Fluoxetine Impact Specific Behaviors Involved in Reproduction , Feeding and Predator Avoidance in the Fish Pimephales Promelas ( Fathead Minnow ). Aquat Toxicol (2014) 151:77–83. doi: 10.1016/j.aquatox.2013.10.012
96. Stoczynski L, van den Hurk P. Effects of Selective Serotonin Reuptake Inhibitor Sertraline on Hybrid Striped Bass Predatory Behavior and Brain Chemistry. Aquat Toxicol (2020) 226:105564. doi: 10.1016/j.aquatox.2020.105564
97. Pérez-Sánchez J, Simó-Mirabet P, Naya-Català F, Martos-Sitcha JA, Perera E, Bermejo-Nogales A, et al. Somatotropic Axis Regulation Unravels the Differential Effects of Nutritional and Environmental Factors in Growth Performance of Marine Farmed Fishes. Front Endocrinol (Lausanne) (2018) 9:687. doi: 10.3389/fendo.2018.00687
98. Wood AW, Duan C, Bern HA. Insulin-Like Growth Factor Signaling in Fish. Int Rev Cytol (2005) 243:215–85. doi: 10.1590/198053143674
99. Reinecke M, Björnsson BT, Dickhoff WW, McCormick SD, Navarro I, Power DM, et al. Growth Hormone and Insulin-Like Growth Factors in Fish: Where We are and Where to Go. Gen Comp Endocrinol (2005) 142:20–4. doi: 10.1016/j.ygcen.2005.01.016
100. Chang JP, Wong AOL. Growth Hormone Regulation in Fish: A Multifactorial Model With Hypothalamic, Peripheral and Local Autocrine/Paracrine Signals. In: Fish Neuroendocrinology, Volume 28 Fish Physiology, Elsevier Inc San Diego (2009) 28:151–95. doi: 10.1016/s1546-5098(09)28004-6
101. Tuomisto J, Mannisto P. Neurotransmitter Regulation of Anterior Pituitary Hormones. Pharmacol Rev (1985) 37:249–332.
102. Chang JP, Marchant TA, Cook AF, Nahorniak CS, Peter RE. Influences of Catecholamines on Growth Hormone Release in Female Goldfish, Carassius Auratus. Neuroendocrinology (1985) 40:463–70. doi: 10.1159/000124116
103. Wong AOL, Chang JP, Peter RE. In Vitro and In Vivo Evidence That Dopamine Exerts Growth Hormone- Releasing Activity in Goldfish. Am J Physiol - Endocrinol Metab (1993) 264:925–32. doi: 10.1152/ajpendo.1993.264.6.e925
104. Chang JP, Abele JT, Van Goor F, Wong AOL, Neumann CM. Role of Arachidonic Acid and Calmodulin in Mediating Dopamine D1- and GnRH-Stimulated Growth Hormone Release in Goldfish Pituitary Cells. Gen Comp Endocrinol (1996) 102:88–101. doi: 10.1006/gcen.1996.0050
105. Mommsen TP, Vijayan MM, Moon TW. Cortisol in Teleosts : Dynamics, Mechanisms of Action , and Metabolic Regulation. Rev Fish Biol Fish (1999) 9:211–68. doi: 10.1023/A:1008924418720
106. Faught E, Vijayan MM. Mechanisms of Cortisol Action in Fish Hepatocytes. Comp Biochem Physiol Part - B Biochem Mol Biol (2016) 199:136–45. doi: 10.1016/j.cbpb.2016.06.012
107. Wendelaar Bonga S. The Stress Response in Fish. Physiol Rev (1997) 77:591–625. doi: 10.1152/physrev.1997.77.3.591
108. Nesan D, Vijayan MM. Maternal Cortisol Mediates Hypothalamus-Pituitary-Interrenal Axis Development in Zebrafish. Sci Rep (2016) 6:22582. doi: 10.1038/srep22582
109. Bouyoucos IA, Schoen AN, Wahl RC, Anderson WG. Ancient Fishes and the Functional Evolution of the Corticosteroid Stress Response in Vertebrates. Comp Biochem Physiol -Part A Mol Integr Physiol (2021) 260:111024. doi: 10.1016/j.cbpa.2021.111024
110. Alsop D, Vijayan MM. Development of the Corticosteroid Stress Axis and Receptor Expression in Zebrafish. Am J Physiol Regul Integr Comp Physiol (2008) 294:R711–9. doi: 10.1152/ajpregu.00671.2007
111. Vijayan MM, Aluru N, Leatherland JF. Stress Response and the Role of Cortisol. Fish Dis Disord (2010) 2:182–201. doi: 10.1079/9781845935535.0182
112. Russell G, Lightman S. The Human Stress Response. Nat Rev Endocrinol (2019) 15:525–34. doi: 10.1038/s41574-019-0228-0
113. Iwama GK, McGeer JC, Wright PA, Wilkie MP, Wood CM. Divalent Cations Enhance Ammonia Excretion in Lahontan Cutthroat Trout in Highly Alkaline Water. J Fish Biol (1997) 50:1061–73. doi: 10.1111/j.1095-8649.1997.tb01630.x
114. Faught E, Vijayan MM. The Mineralocorticoid Receptor is Essential for Stress Axis Regulation in Zebrafish Larvae. Sci Rep (2018) 8:1–11. doi: 10.1038/s41598-018-36681-w
115. Faught E, Vijayan MM. Loss of the Glucocorticoid Receptor in Zebrafish Improves Muscle Glucose Availability and Increases Growth. Am J Physiol - Endocrinol Metab (2019) 316:E1093–104. doi: 10.1152/ajpendo.00045.2019
116. Faught E, Vijayan M. Glucocorticoid and Mineralocorticoid Receptor Activation Modulates Postnatal Growth. J Endocrinol (2020) 244:261–71. doi: 10.1530/JOE-19-0358
117. Andrews MH, Matthews SG. Programming of the Hypothalamo – Pituitary – Adrenal Axis : Serotonergic Involvement. Stress (2004) 7:15–27. doi: 10.1080/10253890310001650277
118. Jiang X, Wang J, Luo T, Li Q. Impaired Hypothalamic-Pituitary-Adrenal Axis and its Feedback Regulation in Serotonin Transporter Knockout Mice. Psychoneuroendocrinology (2009) 34:317–31. doi: 10.1016/j.psyneuen.2008.09.011
119. Winberg S, Nilsson A, Hylland P, So V. Serotonin as a Regulator of Hypothalamic-Pituitary-Interrenal Activity in Teleost Fish. Neurosci Lett (1997) 230:113–6. doi: 10.1016/S0304-3940(97)00488-6
120. Höglund E, Balm PHM, Winberg S. Stimulatory and Inhibitory Effects of 5-HT1A Receptors on Adrenocorticotropic Hormone and Cortisol Secretion in a Teleost Fish, the Arctic Charr (Salvelinus Alpinus). Neurosci Lett (2002) 324:193–6. doi: 10.1016/S0304-3940(02)00200-8
121. Gesto M, Lopez-Patino MA, Hernandez J, Soengas JL, Miguez JM. Gradation of the Stress Response in Rainbow Trout Exposed to Stressors of Different Severity: The Role of Brain Serotonergic and Dopaminergic Systems. J Neuroendocrinol (2015) 27:131–41. doi: 10.1111/jne.12248
122. Lepage O, Tottmar O, Winberg S. Elevated Dietary Intake of L-Tryptophan Counteracts the Stress-Induced Elevation of Plasma Cortisol in Rainbow Trout (Oncorhynchus Mykiss). J Exp Biol (2002) 205:3679–87. doi: 10.1242/jeb.205.23.3679
123. Lim JE, Porteus CS, Bernier NJ. General and Comparative Endocrinology Serotonin Directly Stimulates Cortisol Secretion From the Interrenals in Goldfish. Gen Comp Endocrinol (2013) 192:246–55. doi: 10.1016/j.ygcen.2013.08.008
124. Pepels PPLM, van Helvoort H, Wendelaar Bonga SE, Balm PHM. Corticotropin-Releasing Hormone in the Teleost Stress Response: Rapid Appearance of the Peptide in Plasma of Tilapia (Oreochromis Mossambicus). J Endocrinol (2004) 180:425–38. doi: 10.1677/joe.0.1800425
125. Rotllant J, Ruane NM, Dinis MT, Canario AVM, Power DM. Intra-Adrenal Interactions in Fish: Catecholamine Stimulated Cortisol Release in Sea Bass (Dicentrarchus Labrax L.). Comp Biochem Physiol - A Mol Integr Physiol (2006) 143:375–81. doi: 10.1016/j.cbpa.2005.12.027
126. Olivereau M, Olivereau J. Localization of CRF-Like Immunoreactivity in the Brain and Pituitary of Teleost Fish. Peptides (1988) 9:13–21. doi: 10.1016/0196-9781(88)90004-6
127. Vera-Chang MN, Moon TW, Trudeau VL. Ancestral Fluoxetine Exposure Sensitizes Zebrafish to Venlafaxine-Induced Reductions in Cortisol and Spawning. Endocrinology (2019) 160:2137–42. doi: 10.1210/en.2019-00281
128. Ampatzis K, Dermon CR. Sexual Dimorphisms in Swimming Behavior, Cerebral Metabolic Activity and Adrenoceptors in Adult Zebrafish ( Danio Rerio ). Behav Brain Res (2016) 312:385–93. doi: 10.1016/j.bbr.2016.06.047
Keywords: hormones, reproduction, growth, stress response, monoamines, steroids, pharmaceutical, municipal wastewater effluent
Citation: Thompson WA and Vijayan MM (2022) Antidepressants as Endocrine Disrupting Compounds in Fish. Front. Endocrinol. 13:895064. doi: 10.3389/fendo.2022.895064
Received: 12 March 2022; Accepted: 19 April 2022;
Published: 16 June 2022.
Edited by:
Vance L. Trudeau, University of Ottawa, CanadaReviewed by:
Marilyn N Vera-Chang, Canadian Nuclear Laboratories (CNL), CanadaCopyright © 2022 Thompson and Vijayan. This is an open-access article distributed under the terms of the Creative Commons Attribution License (CC BY). The use, distribution or reproduction in other forums is permitted, provided the original author(s) and the copyright owner(s) are credited and that the original publication in this journal is cited, in accordance with accepted academic practice. No use, distribution or reproduction is permitted which does not comply with these terms.
*Correspondence: Mathilakath M. Vijayan, matt.vijayan@ucalgary.ca
Disclaimer: All claims expressed in this article are solely those of the authors and do not necessarily represent those of their affiliated organizations, or those of the publisher, the editors and the reviewers. Any product that may be evaluated in this article or claim that may be made by its manufacturer is not guaranteed or endorsed by the publisher.